In vitro experimental models of mesothelioma revisited
Introduction
Malignant pleural mesothelioma (MPM) is the most common type of mesothelioma and remains an incurable malignancy with few treatment options. Since 2003 when cisplatin and pemetrexed combination therapy was validated as the standard drug regimen for clinical treatment (1), there has been minimal improvement in long-term survival. Incremental progress has been achieved in eligible MPM patients with the recent addition of bevacizumab (2) to the standard two-drug paradigm, but even this newer combination regimen lacks a strong specific biologic rationale against MPM. Perhaps a systematic reappraisal of the current oncogenesis models for MPM is warranted to refocus research efforts aimed at identifying critical molecular pathways. For the purpose of this review, specific attention is given to analysis of in vitro transformation models of MPM that have provided insights into the various molecular mechanisms and genetic alterations at the core of the MPM malignant state. With a critical understanding of the pros and cons of each in vitro model scheme, principles can be derived to guide future and ongoing research towards a consistent and more physiologically accurate explanation of MPM oncogenesis.
Clinical trends
MPM is an aggressive cancer that arises from the mesothelial lining of the pleura, peritoneum and pericardium, and rarely from the tunica vaginalis of the testis (3). Approximately 80% of mesothelioma cases are pleural in origin and are defined as MPM (4). MPM is highly associated with occupational exposure to asbestos fibers which are widely accepted as the primary causative agent (5). Although asbestos has been nationally banned in many developed regions of the world including Europe, Scandinavia, United Kingdom, Japan, and Australia current notable exceptions exist such as China, Russia, India, Brazil, Canada and the United States (6). Of further note, Canada has implemented legislation to ban asbestos use by 2018 (7). In the United States significant asbestos abatement via regulatory actions of the Environmental Protection Agency have served to limit asbestos exposure as much as practical to the general public (8).
Subsequently, the incidence and prevalence of MPM continue to show alarming trends worldwide. In the United States, for example, even though predictions suggested that the incidence of MPM should have peaked in the early 2000s (9), the incidence rate per 100,000 people, shows no change since 1975 (10). Currently, the annual incidence of MPM in the United States remains approximately 3,200 individuals affected (11). Further, the latest analysis from the Centers for Disease Control and Prevention observed that the annual number of deaths from mesothelioma continued to unexpectedly increase by 4.8% (P for linear time trend <0.001) overall to 2,579 cases in 2015. And the underlying main factor for this rise was increased deaths among persons aged ≥85 years (8). In other countries, current predictions of MPM incidence have not yet reached peak levels. By 2020–2030 and beyond, in industrialized nations alone, the increase is likely to affect thousands of people (12). For the foreseeable future, the prevalence of MPM remains a significant cancer type and the incidence continues to increase worldwide, making mesothelioma a major international health problem (12,13).
Pathogenesis
MPM is a highly complex tumor at the genetic level lacking consistent molecular patterns that would inform on obvious treatment approaches, but which suggest there are multiple active oncogenic programs cooperating to drive this malignancy (14,15). To date, much of the new therapeutic approaches that have been tested in clinical trials have focused intervention efforts on one biological pathway or molecule that is deemed critical to the development and ongoing growth of MPM. Therefore, part of the reason why modern treatments fail to yield a durable cure is because the biological basis of the disease is not fully understood.
In the latest summaries (5,14,16), a current accepted view of MPM pathogenesis derives from in vitro studies of human and murine cells, in vivo murine models, as well as indirect evidence from human surgical or autopsy studies. In brief, MPM oncogenesis is hypothesized to consist of the following major steps: (I) a fraction of inhaled asbestos fibers preferentially reach distal lung alveoli based on particle shape and size characteristics; (II) asbestos fibers transit to the pleural space where drainage mechanisms of stomata (μm in size) emptying into a lymphatic network act to clear the fibers out of the chest; (III) the fibers, because of their shape and size, get retained at stomata on the parietal pleural surface; (IV) macrophages migrate to the pleural space and attempt to phagocytose these fibers, but since they cannot be internalized completely, frustrated phagocytosis ensues to form “black spots” initiating a pro-inflammatory cascade of chemical mediators (reactive oxygen species, cytokines, and growth factors) and likely multiple signaling pathways cooperate to promote oncogenesis; (V) in parallel, there is direct interaction of asbestos fibers with human mesothelial cells (HMC) which incur genotoxic effects and undergo damage necrosis, further perpetuating a cascade of chronic inflammation primarily at the parietal pleura; (VI) presumably, in some parietal HMC, there is enough inherent or acquired resistance to asbestos effects (apoptosis/cell death) that is mediated by nuclear factor-kappa B (NF-κB) signaling, to foster their survival and proliferation (17,18); (VII) in the setting of ongoing asbestos-induced deoxyribonucleic acid (DNA) damage, genetic abnormalities accumulate in those surviving HMC; and finally (VIII) asbestos-induced genetic instability in parietal HMC culminate in a multi-step process of cancer evolution.
A highly controversial topic of MPM pathogenesis concerns the role of simian virus 40 (SV40). While SV40 large-T antigen (Tag) directly interacts with transformation-related protein 53 gene (p53) and retinoblastoma (Rb) tumor suppressor pathways in MPM cells (19), and has been used as a co-factor with asbestos fibers in numerous in vitro (reviewed here in our article) and small animal studies of mesothelial cellular transformation, its direct relevance in human MPM specimens has been strongly questioned. Many human epidemiologic studies performed to date do not support any clinically relevant association between SV40 and human MPM (20). Multiple research efforts (small and multi-institutional groups) have been unable to convincingly detect SV40 genetic sequences in human MPM (21-25). Perhaps more notably, ongoing updates of MPM now have either omitted any further discussion of SV40 as a causative agent (14,16) or suggest that this viral contamination theory should be discarded (5).
Current knowledge gaps
Critical review of the data supporting the current oncogenesis framework exposes persisting assumptions in our knowledge that contribute to unsolved mechanistic links. A few areas of knowledge gaps regarding MPM pathobiology are highlighted:
- It remains unknown how airborne asbestos fibers traverse the lung interstitium to preferentially affect the pleura and incite MPM as opposed to primarily causing disease in lung parenchyma. The lymphatic network draining into stomata on parietal pleura has only been observed in small animal models, never conclusively demonstrated in humans (16,26). Additionally, there is virtually no knowledge about the kinetics of asbestos fiber translocation and deposition in human pleura (27);
- While the majority of studies assume the cell of origin for MPM is the pleural mesothelial cell, a small body of literature theorizes other cell types could be responsible such as mesothelial progenitor cells that influx to parietal pleura or are induced in parietal regions of cellular damage caused by asbestos (5,28);
- Related to this concept is the controversial field of cancer stem cell biology applied to solid tumors for which there is a paucity of literature to support this notion in MPM (29-32). These reports are hampered by lack of a consistent and specific stem cell marker(s), and lack of reproducibility from serial dilution experiments of primary MPM samples;
- Although it is recognized that inflammation plays a role in MPM pathogenesis, the direct molecular mechanism(s), if any, linking inflammation to cancer development have not been described in detail. It is well-accepted that NF-κB generally mediates survival from asbestos-induced effects (cell killing) in some, not all HMC; and it is those surviving HMC which go on to form a tumor (17,18). The mechanism(s) that influence selection of HMC sub-population(s) that can survive the initial killing induced by asbestos remains unknown;
- The early molecular events through which asbestos causes mesothelial cell transformation have yet to be fully understood. Possible mechanisms have been proposed for the pathogenesis of MPM that describe effects on asbestos-exposed HMC and macrophages as: (i) generation of reactive oxygen species, leading to DNA damage and chromosomal alterations that are the basis for development of malignant cells (33); (ii) activation of multiple receptor tyrosine kinases with constitutive proliferation, for example, via epidermal growth factor receptor etc. (34); and (iii) secretion of high mobility group protein B1 (HMGB1) from damaged HMC (although it is not well explained why this is specific to HMC undergoing damage necrosis nor why this is responsible for major effects as multiple other damage-associated molecules are released) to perpetuate a chronic auto-inflammatory cascade via toll-like receptors and receptor for advanced glycation end products (35). But little is understood in terms of which mechanism is activated preferentially by asbestos exposure, nor which of these mechanisms have a primary role during any time points of cellular transformation;
- Also, it cannot be explained in the current framework how MPM progresses to cover an enormous surface area spreading out laterally. Why does MPM not grow and enlarge as a spheroid volume similar to all other solid tumors? A possible hypothesis to partly explain this characteristic would require multiple metachronous foci of mesothelial cells acquiring malignant growth, but direct evidence to explain this phenomenon is unavailable;
- Recent data indicates a polyclonal cell origin for MPM (36). This observation could explain clinical experience that recognizes MPM behaving as a composite of multiple tumors with inter- and intratumor genetic heterogeneity (14,16), contributing to the ability of MPM to generally resist all modes of therapy. But overall, the impact of this finding on MPM biology requires more investigation;
- There are few consistent and highly recurrent genetic mutations acknowledged as oncogenic drivers despite a multitude of chromosomal and genetic MPM profiling studies (14,37). In terms of somatic alterations, the overall mutational burden of MPM is low among various solid tumors most comparable to neuroblastomas (38,39), and, to date, only three driver genes [cyclin-dependent kinase inhibitor 2A gene (CDKN2A), neurofibromin 2 gene (NF2), and BRCA1 associated protein-1 gene (BAP1)] are commonly recognized (40).
Remarkably, the notion of chronic inflammation initiated by asbestos remains a hypothesis without direct human (in vivo) mechanisms delineated (16). Reliable inhalation studies with well-characterized aerosols of various asbestos fibers in long-term exposure animal models have not been reported due to cost, complexity, and lack of specialized instrumentation. Thus, few, if any, in vivo human mechanistic studies confirm the widely held concept of chronic inflammation associated with asbestos (27). Largely, it is indirect evidence that supports the pervasive role of inflammation in MPM pathogenesis. Elevated interleukin (IL)-6 levels in serum and pleural effusions of patients with MPM have been observed to generally support involvement of an inflammatory condition associated with malignancy in the context of asbestos (41,42). Also, pathologic analyses of MPM generally support the notion of intense inflammatory infiltrating cells of the tumor microenvironment in resected specimens (43).
Basis of knowledge gaps
Multiple factors collude to obscure the precise pathogenetic mechanisms specific to MPM. Likely what is required for a comprehensive understanding of the pathologic processes in MPM (or any cancer) is a synthesis of in vitro, in vivo (animal), and human modeling data sets. While current technology does not yet facilitate such a seamless integration of modeling systems, much complementary information can still be curated from each. Tumor tissue specimens represent a diverse and complex mixture of malignant cells and other cell types that, en masse, have achieved multiple milestones characteristic of cancer. Despite increasingly sophisticated technologies to assess genetic alterations of the whole tumor mass, the results may reflect relatively late, advanced cancer mass changes not easily treated by systemic chemotherapeutics or molecular targeted agents. The next alternative to better assess and dissect cancer processes is to use animal models that can reveal the complexity of tumorigenic processes in a living system, however visualization of molecular or biologic events step-by-step and quantitation of data is typically a prohibitive technical challenge. Additionally, extrapolating animal model responses to human cancer patients has yet to fully realize direct translational successes (44). A complementary and still useful type of system is the classical in vitro model of cultured cell lines which permit direct manipulation and precise dissection of gene regulatory networks and predominant signaling pathways (45). In fact, the selection of an appropriate and physiologically relevant in vitro model is important for the investigation of chromosomal changes, epigenetics, initiation and progression, and deregulation of apoptosis and proliferation, etc. However, in vitro systems have many limitations including: lack of cellular heterogeneity/complexity similar to the original tumor, or genotypic and phenotypic drifting away from the original tumor after prolonged culture time, etc. (46). Perhaps a review of MPM in vitro models could suggest further experimental designs that better address some of the knowledge gaps in MPM pathobiology as mentioned here.
In vitro models of oncogenesis
Since the mechanism(s) underlying development of MPM continue to be elucidated, there is no consensus on an ideal in vitro model. Many groups have sought to propose in vitro models of MPM development, although each has pros and cons in their design and execution (Table 1).
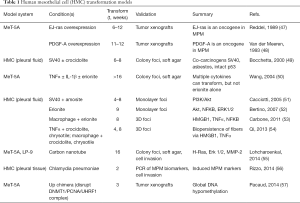
Full table
Transformation by gene transfer
The first in vitro model of MPM oncogenesis as reported by Reddel et al. was created by transfecting guanosine-5'-triphosphate-bound isoform of p21 ras (constitutively activated) gene (EJ-ras) oncogene into an immortalized HMC line MeT-5A (47). The EJ-ras transfected cells were tumorigenic, able to form tumors in nude mice, while untransfected MeT-5A cells did not form tumors. Prior to this work, it was known that primary HMC did not similarly transform with EJ-ras transfection (58), so these authors deduced that multiple, instead of single, molecular steps are likely required by HMC before producing a malignant phenotype. The MeT-5A cells, derived from pleural mesothelium, were immortalized by insertion of Tag (59) which inhibits both p53 and Rb pathways likely providing a permissive genetic background for full cellular transformation to a malignant phenotype. Implicit in this model is the assumption of HMC being the cell of origin for MPM. Along this same conceptual framework of constitutive oncogene(s) being responsible for HMC transformation and oncogenesis, Van der Meeren et al. transfected MeT-5A cells with platelet-derived growth factor-A gene which formed tumors in nude mice allowing them to speculate about the role of autocrine growth signaling in tumorigenesis as well as the possibility of multiple unique pathways leading to the malignant state in MPM (48).
Transformation by asbestos exposure
Early experience by Lechner et al. with exposing HMC to asbestos revealed that HMC were highly and uniquely sensitive to the cytotoxic effects of all asbestos fiber forms tested (60). Despite different concentrations of amosite, mice xenograft tumorigenicity experiments were unsuccessful because most exposed HMC were killed and only rare sub-populations of HMC could be passaged for further study. The few surviving cells from this experimental model did reveal that asbestos induced complex chromosomal aberrations. Thus, this early study, among other similar ones (61), established the apparent paradox of asbestos action: the killing of most HMC upon their exposure yet was the only specific agent known to induce tumorigenicity in certain HMC and ultimately cause MPM. A follow-up study by Gabrielson et al. exposed HMC, MPM cell lines, or previously transformed (tumorigenic) HMC to amosite and observed similar patterns of differential susceptibility to asbestos cytotoxicity, leading them to propose that tumorigenicity and asbestos resistance are independent processes that contribute to the overall process of MPM oncogenesis (62).
Using a different approach, Bocchetta et al. demonstrated that SV40 and asbestos act synergistically as co-carcinogens in soft agar foci formation assays of exposed HMC over a prolonged period of 6 to 8 weeks (49). Interestingly, HMC transfected with a SV40 construct expressing both of its Tag and small tumor antigen were able to transform without crocidolite exposure, but it was the combination of asbestos and SV40 that produced the most foci. This oncogenesis model was developed during the period that SV40 involvement in MPM was a popular concept. Along similar assumptions, Cacciotti et al. demonstrated that SV40 Tag induced, in HMC, survival from asbestos-induced cytotoxicity and apoptosis via phosphatidylinositol-3 kinase (PI3K)/protein kinase B (Akt) signaling (51). This study helped to reinforce the hypothesis of multiple Tag-dependent survival pathways [e.g., hepatocyte growth factor/hepatocyte growth factor receptor (c-Met)] in HMC driving the selection of a sub-population able to evolve to a fully malignant state after they are transformed by asbestos (63).
Transformation by silicates
Others investigated the transforming effects of alternative non-asbestos materials in oncogenic models of MPM, namely because of the known cytotoxic effects of asbestos to HMC paradoxically limiting their formation of transformed tumorigenic cells. Wang et al. explored the ability of combinatorial exposures of inflammatory cytokines [IL-1β and/ or tumor necrosis factor (TNF)-α] with or without erionite to induce transformation of MeT-5A cells as measured by anchorage-independent growth in soft agar assays over prolonged periods of at least 16 weeks (50). Erionite is a silicon-based asbestiform fiber belonging to the mineral class of zeolites found to be naturally occurring in rock formations throughout worldwide locations with known epidemiologic link to MPM (53). Although it is accepted that erionite is a carcinogen (64,65), the molecular mechanism(s) responsible for its toxicity remain obscure with recent recognition that iron, previously thought to be the critical element driving carcinogenicity (66), is not even part of the erionite crystal structure (67). To date, the work from Wang et al. is the only study to demonstrate that prolonged exposure to cytokines (IL-1β with TNF-α) could induce transformation of non-cancer cells without asbestos. Erionite exposure alone did not induce transformation, but required the additive effects of these cytokines.
Contrary results were obtained by Bertino et al. who induced transformation of HMC by low doses of erionite over long-term exposure, noting that this mineral fiber was poorly cytotoxic but able to stimulate pathologic proliferation via constitutive signaling of Akt, NF-κB and extracellular signal-regulated kinases (Erk) 1/2 pathways (52). Amosite and chrysotile asbestos fibers were not able to transform HMC under the same experimental conditions, but, like other studies, did produce extensive cell killing by cytotoxicity. It was claimed that these transformation results were not dependent on the presence of SV40, yet there were no confirmatory assays specifically checking for SV40.
Transformation by co-culture with macrophages
Carbone et al. sought to clarify the biologic effects of erionite in HMC by co-culturing with macrophages in a dual chamber set-up that mimicked (proposed in vivo events) the process of inflammatory cell recruitment and activation at sites of fiber deposition (53). After a prolonged culture period, 3D foci developed, consistent with their interpretation of cellular transformation, in only those erionite-exposed HMC with macrophages but not in unexposed HMC with macrophages. Erionite fibers exerted effects on both HMC and macrophages culminating in a self-amplifying cascade of programmed cell necrosis and chronic inflammation. This same research group followed-up this experimental design of in vitro MPM oncogenesis using more combinations of co-culture conditions among HMC, macrophages, cytokines, and asbestos fiber types (crocidolite or chrysotile) (54). They showed that HMC required TNF-α to survive asbestos-induced cytotoxicity and that either fiber could transform HMC as observed in 3D foci formation assay. When HMC were exposed solely to either asbestos, they released HMGB1 and TNF-α in a fiber-density dependent manner, but no cells survived past 2 weeks and hence no transformation occurred under these conditions. Pretreating the HMC with TNF-α reduced asbestos cytotoxicity. Asbestos exposure of HMC co-cultured with macrophages, which secrete presumably enough TNF-α to protect HMC from necrosis, demonstrated transformation after about 8 weeks.
Transformation by other agents
Technological advances have inadvertently developed high aspect ratio engineered nanoparticles, such as single-walled carbon nanotube (SWCNT), which share physical characteristics to asbestos fibers leading to concerns that these newly manmade materials could cause MPM (26,68). Lohcharoenkal et al. recently demonstrated in Met-5A cells exposed to prolonged SWCNT doses, the formation of increased and large-sized colonies in soft agar as well as invasiveness by transwell migration consistent with a transformed phenotype (55). However, LP-9 peritoneal normal mesothelial cells (69), only showed modest colony formation yet did exhibit similar aggressive changes in invasiveness when induced with SWCNT. In additional functional assays of both MeT-5A and LP-9 cells, upregulated transforming protein p21 gene (H-Ras), Erk 1/2, and matrix metalloproteinase-2 (MMP-2) signaling were attributed to SWCNT induced changes. Since SWCNT continue to find wider usage in all fields of electronics, optics, energy storage, and/or alloys to name some of the major applications, this material could represent another future threat for an increase in MPM cases (70).
An alternative new concept regarding the pathogenesis of MPM that has not gained traction involves the purported role of Chlamydia pneumoniae as discussed by Rizzo et al. (56). They infected primary HMC with the bacteria and relied on induced expression of MPM biomarkers calretinin, Wilms’ tumor 1, and osteopontin as a surrogate measure of cellular transformation. Aside from not providing definitive evidence of transformed cells (anchorage-independent growth assays or tumor formation in mice), the interaction, if any, with asbestos is not discussed nor is there a compelling human epidemiologic link established. Another more intriguing concept was reported by Pacaud et al. who showed that global DNA hypomethylation induced by the UP chimera protein disrupting a DNA methyltransferase complex deoxyribonucleic acid (cytosine-5)-methyltransferase 1 (DNMT1)/proliferating cell nuclear antigen (PCNA)/ubiquitin-like, containing PHD and RING finger domains, 1 (UHRF1), could transform MeT-5A cells to produce tumors in mice (57). This model is one of the first to demonstrate the impact of epigenetic dysregulation in tumorigenesis of HMC cells. As this study was investigating pan-cancer effects, they did not pursue a direct link of this oncogenic mechanism to asbestos effect. Epigenetic dysfunction could explain MPM cases without apparent asbestos exposure although the inciting mechanisms remain to be elucidated.
Limitations of mesothelioma models
Over about 30 years, numerous in vitro oncogenesis models have been proposed that reflect the increased understanding in MPM pathobiology and incorporate recently recognized molecular mechanisms. Nevertheless, there remains missing knowledge concerning the precise steps that link asbestos exposure to the selection for resistance to cytotoxicity as the HMC integrates these multiple molecular perturbations to complete cell transformation and also in how genetic alterations accumulate to produce a committed malignant cell. These areas of ambiguity culminate in lack of consensus on a universally accepted in vitro model of MPM oncogenesis.
Most of these in vitro MPM models (Table 1) observed foci formation of transformed cells usually only after lengthy time intervals, ranging at least 4 to 16 weeks. The efficiency of induced cells to transform is relatively low with many cells being eliminated because of asbestos-induced cytotoxicity when the exposure conditions are not carefully calibrated. Another limit is lack of a precise definition for cell transformation, but most commonly either anchorage-independent cell growth and/or xenograft tumor formation should be demonstrated. Unfortunately, there are even ambiguous descriptions of what can constitute anchorage-independent cell growth as demonstrated in this review. While soft-agar growth is well-accepted, other assays such as monolayer foci and 3D foci remain ambiguous as to the precise growth conditions (Table 1). Additionally, the baseline cells are varied and none of them are truly “normal”. MeT-5A cells are altered with SV40 sequences and have abnormal ploidy status due to long-term adaptation in cell culture (our unpublished data). LP-9 cells too easily senesce and, in general, grow very slowly, making cultivation of sufficient numbers of cells for ongoing in vitro use inconsistent. Also, there is a notion of differential biology between pleural and peritoneal HMC in responding to asbestos effects (71) that may affect interpretation of oncogenesis mechanisms. Even primary HMC cells (from fluids of the pleural or pericardial spaces) can adapt away from their native in vivo state as part of the thin mesothelium, possibly biasing their behavior to transformation conditions since these HMC have adapted to non-contact growth in pleural fluid unattached to the sub-mesothelial layers.
Another perplexing aspect of MPM in vitro models is the simultaneous requirement for inflammatory priming of non-malignant cells in the presence of asbestos. Under this condition, it is difficult to reconcile and discern the precise sequence of survival signaling since NF-κB can activate a large number of downstream genes and pathways in a cell-type and context-dependent manner (72,73). Additional insights into the pathophysiology of MPM genesis could be discerned if the specific NF-κB-activated gene networks could be identified. With inflammation and asbestos effects exerted simultaneously, this may not be an effective method to delineate detailed sequences of molecular events necessary for full cellular transformation (i.e., tumorigenicity).
Conclusions
For the foreseeable future, MPM will remain a significant worldwide malignancy without effective interventions that translate into long-term survival for the majority of affected patients. Despite caveats, in vitro oncogenesis models have contributed important insights for a better understanding of MPM pathobiology. There remain molecular mechanisms to be resolved in greater detail at various steps of the current accepted model of MPM development that incorporates asbestos genotoxic and mutagenic effects with inflammatory signals. It will be of great interest to see further innovation in different in vitro model schemes that could yield more information. Recent studies reviewed here suggest several upcoming novel insights into pathogenic mechanisms of MPM, for example, regarding the role of epigenetics and other causative agents.
Acknowledgements
The authors thank Nisan Bhattacharyya, PhD for his assistance with collecting references and assembling this manuscript.
Footnote
Conflicts of Interest: The authors have no conflicts of interest to declare.
References
- Vogelzang NJ, Rusthoven JJ, Symanowski J, et al. Phase III study of pemetrexed in combination with cisplatin versus cisplatin alone in patients with malignant pleural mesothelioma. J Clin Oncol 2003;21:2636-44. [Crossref] [PubMed]
- Zalcman G, Mazieres J, Margery J, et al. Bevacizumab for newly diagnosed pleural mesothelioma in the Mesothelioma Avastin Cisplatin Pemetrexed Study (MAPS): a randomised, controlled, open-label, phase 3 trial. Lancet 2016;387:1405-14. [Crossref] [PubMed]
- Carbone M, Ly BH, Dodson RF, et al. Malignant mesothelioma: facts, myths, and hypotheses. J Cell Physiol 2012;227:44-58. [Crossref] [PubMed]
- Delgermaa V, Takahashi K, Park EK, et al. Global mesothelioma deaths reported to the World Health Organization between 1994 and 2008. Bull World Health Organ 2011;89:716-24, 724A-724C.
- Røe OD, Stella GM. Malignant pleural mesothelioma: history, controversy and future of a manmade epidemic. Eur Respir Rev 2015;24:115-31. [Crossref] [PubMed]
- Bahk J, Choi Y, Lim S, et al. Why some, but not all, countries have banned asbestos. Int J Occup Environ Health 2013;19:127-35. [Crossref] [PubMed]
- Ruff K. How Canada's Asbestos Industry Was Defeated in Quebec. New Solut 2017;26:543-56. [Crossref] [PubMed]
- Mazurek JM, Syamlal G, Wood JM, et al. Malignant Mesothelioma Mortality - United States, 1999-2015. MMWR Morb Mortal Wkly Rep 2017;66:214-8. [Crossref] [PubMed]
- Price B, Ware A. Time trend of mesothelioma incidence in the United States and projection of future cases: an update based on SEER data for 1973 through 2005. Crit Rev Toxicol 2009;39:576-88. [Crossref] [PubMed]
- Surveillance Research Program of the NCI. SEER Cancer Statistics Review (1975-2013). 2016. Availale online: https://seer.cancer.gov/archive/csr/1975_2013/
- Henley SJ, Larson TC, Wu M, et al. Mesothelioma incidence in 50 states and the District of Columbia, United States, 2003-2008. Int J Occup Environ Health 2013;19:1-10. [Crossref] [PubMed]
- Robinson BM. Malignant pleural mesothelioma: an epidemiological perspective. Ann Cardiothorac Surg 2012;1:491-6. [PubMed]
- Beckett P, Edwards J, Fennell D, et al. Demographics, management and survival of patients with malignant pleural mesothelioma in the National Lung Cancer Audit in England and Wales. Lung Cancer 2015;88:344-8. [Crossref] [PubMed]
- Bononi A, Napolitano A, Pass HI, et al. Latest developments in our understanding of the pathogenesis of mesothelioma and the design of targeted therapies. Expert Rev Respir Med 2015;9:633-54. [Crossref] [PubMed]
- Xu Y, Zheng M, Merritt RE, et al. miR-1 induces growth arrest and apoptosis in malignant mesothelioma. Chest 2013;144:1632-43. [Crossref] [PubMed]
- Mossman BT, Shukla A, Heintz NH, et al. New insights into understanding the mechanisms, pathogenesis, and management of malignant mesotheliomas. Am J Pathol 2013;182:1065-77. [Crossref] [PubMed]
- Sartore-Bianchi A, Gasparri F, Galvani A, et al. Bortezomib inhibits nuclear factor-kappaB dependent survival and has potent in vivo activity in mesothelioma. Clin Cancer Res 2007;13:5942-51. [Crossref] [PubMed]
- Yang H, Bocchetta M, Kroczynska B, et al. TNF-alpha inhibits asbestos-induced cytotoxicity via a NF-kappaB-dependent pathway, a possible mechanism for asbestos-induced oncogenesis. Proc Natl Acad Sci U S A 2006;103:10397-402. [Crossref] [PubMed]
- Carbone M, Rizzo P, Grimley PM, et al. Simian virus-40 large-T antigen binds p53 in human mesotheliomas. Nat Med 1997;3:908-12. [Crossref] [PubMed]
- Weiner SJ, Neragi-Miandoab S. Pathogenesis of malignant pleural mesothelioma and the role of environmental and genetic factors. J Cancer Res Clin Oncol 2009;135:15-27. [Crossref] [PubMed]
- Aoe K, Hiraki A, Murakami T, et al. Infrequent existence of simian virus 40 large T antigen DNA in malignant mesothelioma in Japan. Cancer Sci 2006;97:292-5. [Crossref] [PubMed]
- López-Ríos F, Illei PB, Rusch V, et al. Evidence against a role for SV40 infection in human mesotheliomas and high risk of false-positive PCR results owing to presence of SV40 sequences in common laboratory plasmids. Lancet 2004;364:1157-66. [Crossref] [PubMed]
- Manfredi JJ, Dong J, Liu WJ, et al. Evidence against a role for SV40 in human mesothelioma. Cancer Res 2005;65:2602-9. [Crossref] [PubMed]
- Strickler HD. International SVWG. A multicenter evaluation of assays for detection of SV40 DNA and results in masked mesothelioma specimens. Cancer Epidemiol Biomarkers Prev 2001;10:523-32. [PubMed]
- Ziegler A, Seemayer CA, Hinterberger M, et al. Low prevalence of SV40 in Swiss mesothelioma patients after elimination of false-positive PCR results. Lung Cancer 2007;57:282-91. [Crossref] [PubMed]
- Donaldson K, Murphy FA, Duffin R, et al. Asbestos, carbon nanotubes and the pleural mesothelium: a review of the hypothesis regarding the role of long fibre retention in the parietal pleura, inflammation and mesothelioma. Part Fibre Toxicol 2010;7:5. [Crossref] [PubMed]
- Broaddus VC, Everitt JI, Black B, et al. Non-neoplastic and neoplastic pleural endpoints following fiber exposure. J Toxicol Environ Health B Crit Rev 2011;14:153-78. [Crossref] [PubMed]
- Herrick SE, Mutsaers SE. Mesothelial progenitor cells and their potential in tissue engineering. Int J Biochem Cell Biol 2004;36:621-42. [Crossref] [PubMed]
- Cortes-Dericks L, Carboni GL, Schmid RA, et al. Putative cancer stem cells in malignant pleural mesothelioma show resistance to cisplatin and pemetrexed. Int J Oncol 2010;37:437-44. [PubMed]
- Cortes-Dericks L, Froment L, Boesch R, et al. Cisplatin-resistant cells in malignant pleural mesothelioma cell lines show ALDH(high)CD44(+) phenotype and sphere-forming capacity. BMC Cancer 2014;14:304. [Crossref] [PubMed]
- Ghani FI, Yamazaki H, Iwata S, et al. Identification of cancer stem cell markers in human malignant mesothelioma cells. Biochem Biophys Res Commun 2011;404:735-42. [Crossref] [PubMed]
- Pasdar EA, Smits M, Stapelberg M, et al. Characterisation of mesothelioma-initiating cells and their susceptibility to anti-cancer agents. PLoS One 2015;10:e0119549. [Crossref] [PubMed]
- Huang SX, Jaurand MC, Kamp DW, et al. Role of mutagenicity in asbestos fiber-induced carcinogenicity and other diseases. J Toxicol Environ Health B Crit Rev 2011;14:179-245. [Crossref] [PubMed]
- Pache JC, Janssen YM, Walsh ES, et al. Increased epidermal growth factor-receptor protein in a human mesothelial cell line in response to long asbestos fibers. Am J Pathol 1998;152:333-40. [PubMed]
- Yang H, Rivera Z, Jube S, et al. Programmed necrosis induced by asbestos in human mesothelial cells causes high-mobility group box 1 protein release and resultant inflammation. Proc Natl Acad Sci U S A 2010;107:12611-6. [Crossref] [PubMed]
- Comertpay S, Pastorino S, Tanji M, et al. Evaluation of clonal origin of malignant mesothelioma. J Transl Med 2014;12:301. [Crossref] [PubMed]
- Hiddinga BI, Rolfo C, van Meerbeeck JP. Mesothelioma treatment: Are we on target? A review. J Adv Res 2015;6:319-30. [Crossref] [PubMed]
- Lawrence MS, Stojanov P, Polak P, et al. Mutational heterogeneity in cancer and the search for new cancer-associated genes. Nature 2013;499:214-8. [Crossref] [PubMed]
- Sugarbaker DJ, Richards WG, Gordon GJ, et al. Transcriptome sequencing of malignant pleural mesothelioma tumors. Proc Natl Acad Sci U S A 2008;105:3521-6. [Crossref] [PubMed]
- Bott M, Brevet M, Taylor BS, et al. The nuclear deubiquitinase BAP1 is commonly inactivated by somatic mutations and 3p21.1 losses in malignant pleural mesothelioma. Nat Genet 2011;43:668-72. [Crossref] [PubMed]
- Amati M, Tomasetti M, Mariotti L, et al. Assessment of biomarkers in asbestos-exposed workers as indicators of cancer risk. Mutat Res 2008;655:52-8. [Crossref] [PubMed]
- Nakano T, Chahinian AP, Shinjo M, et al. Interleukin 6 and its relationship to clinical parameters in patients with malignant pleural mesothelioma. Br J Cancer 1998;77:907-12. [Crossref] [PubMed]
- Linton A, van Zandwijk N, Reid G, et al. Inflammation in malignant mesothelioma - friend or foe? Ann Cardiothorac Surg 2012;1:516-22. [PubMed]
- Gould SE, Junttila MR, de Sauvage FJ. Translational value of mouse models in oncology drug development. Nat Med 2015;21:431-9. [Crossref] [PubMed]
- van Staveren WC, Solis DY, Hebrant A, et al. Human cancer cell lines: Experimental models for cancer cells in situ? For cancer stem cells? Biochim Biophys Acta 2009;1795:92-103.
- Domcke S, Sinha R, Levine DA, et al. Evaluating cell lines as tumour models by comparison of genomic profiles. Nat Commun 2013;4:2126. [Crossref] [PubMed]
- Reddel RR, Malan-Shibley L, Gerwin BI, et al. Tumorigenicity of human mesothelial cell line transfected with EJ-ras oncogene. J Natl Cancer Inst 1989;81:945-8. [Crossref] [PubMed]
- Van der Meeren A, Seddon MB, Betsholtz CA, et al. Tumorigenic conversion of human mesothelial cells as a consequence of platelet-derived growth factor-A chain overexpression. Am J Respir Cell Mol Biol 1993;8:214-21. [Crossref] [PubMed]
- Bocchetta M, Di Resta I, Powers A, et al. Human mesothelial cells are unusually susceptible to simian virus 40-mediated transformation and asbestos cocarcinogenicity. Proc Natl Acad Sci U S A 2000;97:10214-9. [Crossref] [PubMed]
- Wang Y, Faux SP, Hallden G, et al. Interleukin-1beta and tumour necrosis factor-alpha promote the transformation of human immortalised mesothelial cells by erionite. Int J Oncol 2004;25:173-8. [PubMed]
- Cacciotti P, Barbone D, Porta C, et al. SV40-dependent AKT activity drives mesothelial cell transformation after asbestos exposure. Cancer Res 2005;65:5256-62. [Crossref] [PubMed]
- Bertino P, Marconi A, Palumbo L, et al. Erionite and asbestos differently cause transformation of human mesothelial cells. Int J Cancer 2007;121:12-20. [Crossref] [PubMed]
- Carbone M, Baris YI, Bertino P, et al. Erionite exposure in North Dakota and Turkish villages with mesothelioma. Proc Natl Acad Sci U S A 2011;108:13618-23. [Crossref] [PubMed]
- Qi F, Okimoto G, Jube S, et al. Continuous exposure to chrysotile asbestos can cause transformation of human mesothelial cells via HMGB1 and TNF-alpha signaling. Am J Pathol 2013;183:1654-66. [Crossref] [PubMed]
- Lohcharoenkal W, Wang L, Stueckle TA, et al. Role of H-Ras/ERK signaling in carbon nanotube-induced neoplastic-like transformation of human mesothelial cells. Front Physiol 2014;5:222. [Crossref] [PubMed]
- Rizzo A, Carratelli CR, De Filippis A, et al. Transforming activities of Chlamydia pneumoniae in human mesothelial cells. Int Microbiol 2014;17:185-93. [PubMed]
- Pacaud R, Brocard E, Lalier L, et al. The DNMT1/PCNA/UHRF1 disruption induces tumorigenesis characterized by similar genetic and epigenetic signatures. Sci Rep 2014;4:4230. [Crossref] [PubMed]
- Tubo RA, Rheinwald JG. Normal human mesothelial cells and fibroblasts transfected with the EJras oncogene become EGF-independent, but are not malignantly transformed. Oncogene Res 1987;1:407-21. [PubMed]
- Ke Y, Reddel RR, Gerwin BI, et al. Establishment of a human in vitro mesothelial cell model system for investigating mechanisms of asbestos-induced mesothelioma. Am J Pathol 1989;134:979-91. [PubMed]
- Lechner JF, Tokiwa T, LaVeck M, et al. Asbestos-associated chromosomal changes in human mesothelial cells. Proc Natl Acad Sci U S A 1985;82:3884-8. [Crossref] [PubMed]
- Xu L, Flynn BJ, Ungar S, et al. Asbestos induction of extended lifespan in normal human mesothelial cells: interindividual susceptibility and SV40 T antigen. Carcinogenesis 1999;20:773-83. [Crossref] [PubMed]
- Gabrielson EW, Van der Meeren A, Reddel RR, et al. Human mesothelioma cells and asbestos-exposed mesothelial cells are selectively resistant to amosite toxicity: a possible mechanism for tumor promotion by asbestos. Carcinogenesis 1992;13:1359-63. [Crossref] [PubMed]
- Cacciotti P, Libener R, Betta P, et al. SV40 replication in human mesothelial cells induces HGF/Met receptor activation: a model for viral-related carcinogenesis of human malignant mesothelioma. Proc Natl Acad Sci U S A 2001;98:12032-7. [Crossref] [PubMed]
- Carthew P, Hill RJ, Edwards RE, et al. Intrapleural administration of fibres induces mesothelioma in rats in the same relative order of hazard as occurs in man after exposure. Hum Exp Toxicol 1992;11:530-4. [Crossref] [PubMed]
- Okayasu R, Wu L, Hei TK. Biological effects of naturally occurring and man-made fibres: in vitro cytotoxicity and mutagenesis in mammalian cells. Br J Cancer 1999;79:1319-24. [Crossref] [PubMed]
- Eborn SK, Aust AE. Effect of iron acquisition on induction of DNA single-strand breaks by erionite, a carcinogenic mineral fiber. Arch Biochem Biophys 1995;316:507-14. [Crossref] [PubMed]
- Gualtieri AF, Gandolfi NB, Pollastri S, et al. Where is iron in erionite? A multidisciplinary study on fibrous erionite-Na from Jersey (Nevada, USA). Sci Rep 2016;6:37981. [Crossref] [PubMed]
- Takagi A, Hirose A, Nishimura T, et al. Induction of mesothelioma in p53+/- mouse by intraperitoneal application of multi-wall carbon nanotube. J Toxicol Sci 2008;33:105-16. [Crossref] [PubMed]
- Connell ND, Rheinwald JG. Regulation of the cytoskeleton in mesothelial cells: reversible loss of keratin and increase in vimentin during rapid growth in culture. Cell 1983;34:245-53. [Crossref] [PubMed]
- Shvedova AA, Kisin ER, Porter D, et al. Mechanisms of pulmonary toxicity and medical applications of carbon nanotubes: Two faces of Janus? Pharmacol Ther 2009;121:192-204. [Crossref] [PubMed]
- Dragon J, Thompson J, MacPherson M, et al. Differential Susceptibility of Human Pleural and Peritoneal Mesothelial Cells to Asbestos Exposure. J Cell Biochem 2015;116:1540-52. [Crossref] [PubMed]
- Dajee M, Lazarov M, Zhang JY, et al. NF-kappaB blockade and oncogenic Ras trigger invasive human epidermal neoplasia. Nature 2003;421:639-43. [Crossref] [PubMed]
- Maeda S, Chang L, Li ZW, et al. IKKbeta is required for prevention of apoptosis mediated by cell-bound but not by circulating TNFalpha. Immunity 2003;19:725-37. [Crossref] [PubMed]