LNCAROD was stabilized through N6-methyladenosine methylation and exerted its anticancer effects in lung squamous cell carcinoma by inhibiting SIRT1 activity via CCAR2
Highlight box
Key findings
• To the best of our knowledge, this study is the first to demonstrate that LNCAROD is specifically and highly expressed in lung squamous cell carcinoma (LUSC) cells and tissues. Consistent with this, we found that LNCAROD expression was mediated by IGF2BP2 N6-methyladenosine methylation, which, along with CCAR2 inhibited, SIRTI1’s acetylation activity. This leads to the inhibition of p53 protein acetylation, which eventually leads to mitochondrial apoptosis in LUSC cells, and the inhibition of cell proliferation, migration and invasion.
What is known and what is new?
• Long noncoding RNAs that regulate gene expression play a crucial role in tumorigenesis and progression.
• We found that LNCAROD could interact with CCAR2 through m6A methylation, inducing CCAR2 to suppress the activity of SIRT1, thereby enhancing the acetylation of the p53 protein.
What is the implication, and what should change now?
• The data presented in this study indicate that LNCAROD figures prominently in the onset and progression of LUSC. LNCAROD could serve as a future target for the development of novel therapeutic strategies.
Introduction
Lung cancer is a malignant tumor with high global morbidity and mortality worldwide (1), with its incidence ranking second only to breast cancer. Lung squamous cell carcinoma (LUSC) accounts for nearly 30% of all histological lung cancer types (2), making it a key pathological subtype. As most cases of LUSC have no clear driver gene, chemotherapy is indispensable in the treatment of patients with nonsurgical advanced LUSC, with tyrosine kinase inhibitor (TKI) drug-targeted therapy being commonly applied in clinical settings (3). However, the etiology and mechanism of resistance to chemotherapy in LUSC remain unclear. Therefore, facilitating a greater understanding of the occurrence and development of LUSC and the changes underlying genetic and epigenetic mechanisms following chemotherapy is necessary for the development of more effective therapeutic interventional drugs. LUSC is a common pathological type of non-small cell lung cancer (NSCLC) (4), but at present, compared to lung adenocarcinoma (LUAD), research on its pathogenesis and treatment progress is lacking.
Long noncoding RNA (lncRNA) constitute a family of RNA with transcripts longer than 200 nucleotides and generally do not have a protein-coding function (5). LncRNAs are involved in epigenetic, transcriptional, and posttranscriptional regulation of gene expression in cells (6) and also participate in several biological processes, including cell growth, differentiation, and apoptosis (7). The expression of lncRNA Hoxa-AS3 in NSCLC is upregulated following cisplatin treatment and its knockdown can enhance the efficacy of cisplatin in vitro and in vivo, thereby reducing the tolerance of cells to cisplatin. In one mechanism study, lncRNA Hoxa-AS3 induced epithelial-mesenchymal transition (EMT) by downregulating the expression of HOXA3 to promote the cisplatin resistance of NSCLC cells (8). In breast cancer, lncRNA SNHG1 promotes macrophage polarization toward the M2 phenotype and tumor growth and angiogenesis by regulating the phosphorylation of STAT6 (9). lncRNA FGD5-AS1 is highly expressed in multiple cancers, and FGD5-AS1 mediates the expression of miR-520a-3p and KIAA1522 as a competing endogenous (ceRNA). Following the upregulation of KIAA1522 expression, KIAA1522 promotes the proliferation and migration of multiple cancers (10).
In eukaryotes, N6-methyladenosine (m6A) is the most abundantly occurring-and most studied and well-defined-mRNA methylation modification (11). LncRNA-CBSLR can interact with YTHDF2, resulting in the activation of the CBSLR/YTHDF2/CBS signaling axis in gastric cancer (12). Moreover, it decreases the stability of CBS messenger RNA (mRNA) through promoting the binding of YTHDF2 to the m6A-modified coding sequence (CDS) of CBS mRNA. Upregulation of CBSLR expression results in chemoresistance both in vitro and in vivo and is positively correlated with poor prognosis and poor chemotherapy efficacy. In gastric cancer, the expression of lncRNA TP53TG1 is downregulated. ALKBH5 mediates the m6A modification of TP53TG1, thereby reducing the stability of ALKBH5 and downregulating its expression. This results in the weakening of the interaction between TP53TG1 and CIP2A and the degradation of CIP2A via ubiquitination, which in turn contributes to the occurrence and development of gastric cancer (13). In colorectal cancer (CRC), hypoxia induces HIF-1α, which mediates transcription and leads to the high expression of lncRNA STEAP3-AS1. STEAP3-AS1 competitively binds to YTHDF2 and promotes the dissociation of YTHDF2 and STEAP3 mRNA. By protecting STEAP3 from m6A-mediated degradation, the upregulation of STEAP3 promotes the proliferation and metastasis of CRC in vitro and in vivo by upregulating Fe2+ and GSK3β phosphorylation and activating the WNT signaling pathway through β-catenin (14). The research on RNA m6A methylation is mainly centered on mRNA, and studies on lncRNA m6A methylation are scarce.
In this study, we identified a lncRNA, LNCAROD, which was found to be specifically expressed in LUSC and associated with poor overall survival and prognosis. In LUSC cells, LNCAROD could interact with CCAR2 through m6A methylation, facilitating CCAR2 in suppressing the activity of SIRT1, leading to enhanced acetylation of the p53 protein. LNCAROD could stabilize and maintain the activity of the CCAR2 protein to promote apoptosis and inhibit cell growth, migration, and invasion. We present this article in accordance with the ARRIVE and MDAR reporting checklists (available at https://tlcr.amegroups.com/article/view/10.21037/tlcr-2025-267/rc).
Methods
Gene set enrichment analysis (GSEA)
The goal of GSEA is to identify gene sets that are associated with particular biological processes or pathways (15). This is achieved by assessing the enrichment of the entire gene set across various biological conditions and by linking genes to predefined gene sets based on alterations in their expression levels. We obtained the h.all.v7.5.1.symbols from the Molecular Signature Database and used GSEA version 4.2.3 software to investigate the biological pathways related to the high or low expression of LNCAROD.
Tissue samples
All experiments involving human samples were reviewed and approved by the Ethics Committee of Sun Yat-sen University (approval No. SL-B2023-296-01). This study was conducted in accordance with the Declaration of Helsinki and its subsequent amendments. Tumor tissues and adjacent nontumor tissues were obtained from patients with LUSC (n=40) at Sun Yat-sen University Cancer Center. After surgical resection, samples were flash frozen in liquid nitrogen and stored in liquid nitrogen. No patient received any antineoplastic therapy before surgery. Preoperative candidates were informed about the study, with routine examinations performed 3 days before surgery. For this type of study, formal consent was not required, as many of the participants died when the study was initiated.
Cell lines
Human LUSC cell lines (SK-MES-1, NCI-H2170, NCI-H1703, and NCI-H520) and human normal lung epithelial cells (BEAS-2B) were purchased from Shanghai FuHeng Biology Co., Ltd. (Shanghai, China). SK-MES-1 cells were grown in minimal essential medium (MEM; Gibco, USA), and NCI-H2170, NCI-H1703, and NCI-H520 cells were grown in RPMI1640 medium (Gibco). BEAS-2B cells were grown in Dulbecco’s Modified Eagle Medium (DMEM; Gibco). All media were supplemented with 10% fetal bovine serum (FBS; NEWZERUM, New Zealand) and penicillin and streptomycin (Gibco). All cells were cultured in a Heracell 150i CO2 Incubator (Thermo Scientific, Waltham, MA, USA) at 37 ℃ in an environment with 5% CO2.
Real-time quantitative polymerase chain reaction (RT-qPCR)
Total RNA was isolated from tissue samples and cells using the TRIzol reagent (Invitrogen, Thermo Fisher Scientific). After removal of trace amounts of genomic DNA from the total RNA sample with dsDNase, 1 µg of RNA was employed for reverse transcription in order to generate complement DNA (cDNA), with the Maxima First Strand cDNA Synthesis Kit (Thermo Fisher Scientific) used to conduct RT-qPCR. Reverse transcription was performed using a random primer for LNCAROD and U6. RT-qPCR was performed CFX96 (Bio-Rad Laboratories, USA), and the reaction system comprised the TB Green Fast qPCR Mix (Takara Bio, Japan). The quantification of changes in RNA expression was performed via the 2-ΔΔCt method, with the primer sequences employed in this investigation detailed in Table 1.
Table 1
Name | Sequence |
---|---|
LNCAROD | F: CATGCTCCATCACATCATCCT |
NR_120641.1 | R: GCTCCAGCATGCAGAGATAA |
LNCAROD | F: AGATGACAGAGTCTAGGTTAGGG |
NR_120642.1 | R: AGTAGCCTGTGTTCCTTCTAAAC |
U6 | F: GCTTCGGCAGCACATATACTA |
NR_004394.1 | R: CGAATTTGCGTGTCATCCTTG |
GAPDH | F: CAAGAGCACAAGAGGAAGAGAG |
NM_002046.7 | R: CTACATGGCAACTGTGAGGAG |
CCAR2 | F: GATGATGGAGAGGAGGAGTTTG |
NM_021174.6 | R: GGCATGTCCTGAAGTGAAGA |
IGF2BP2 | F: AGCAGCAGGAGCAGAAATAC |
NM_006548.6 | R: GGTGTTGGAAGGGCTACATT |
qPCR, quantitative polymerase chain reaction.
Construction of stable cell lines and transient gene expression
Overexpression plasmids and short hairpin (shRNA) constructs used in the study were purchased from GeneCopoeia (Rockville, USA). HEK293T cells were transfected using Hilymax reagent (Dojindo Laboratories, Kumamoto, Japan). Specifically, the target plasmid was cotransfected with helper packaging plasmids (psPAX2 and pMD2.G), and the cell supernatant was collected. After the lentivirus was obtained, SK-MES-1 and NCI-H1703 cells were transfected to establish stable gene-expressing cell lines. Small interfering RNA (siRNA) was synthesized by RiboBio Co., Ltd. (Guangzhou, China). Lipofectamin RNAiMAX (Invitrogen) was used for transfection. The gene sequence numbers, shRNA interference sequences, and siRNA sequences are listed in Table 2.
Table 2
Name | Sequence |
---|---|
shRNA-NC | GTTGAGAGACCGTTGAGTAGA |
LNCAROD-shRNA1 | GCAGTGCAGGTTAATTCAATG |
LNCAROD-shRNA2 | GGCTACTGCGATGAGGATTGT |
LNCAROD-shRNA3 | GCCTGAGTCCTTGTAGACTCT |
IGF2BP2-shRNA1 | GGGTAAAGTGGAATTGCATGG |
IGF2BP2-shRNA2 | GCCGTTGTCAACGTCACATAT |
IGF2BP2-shRNA3 | GAACTACTCCTTCAAGATTTC |
CCAR2-OE | NM_021174.6 |
CCAR2-shRNA1 | GCGGGTCTTCACTGGTATTGT |
CCAR2-shRNA2 | GGTCTTCACTGGTATTGTTAC |
CCAR2-shRNA3 | GCCTCTGAGTCTCTTCCAAAC |
AROS-OE | NM_194326.4 |
AROS-shRNA1 | AGGCTGAGGGCACCGTGTTCA |
AROS-shRNA2 | GGCTGAGGGCACCGTGTTCAC |
AROS-shRNA3 | GCTGAGGGCACCGTGTTCACC |
AROS-siRNA1 | GGCACCGTGTTCACCGAGGAA |
AROS-siRNA2 | GCACCGTGTTCACCGAGGAAG |
siRNA-NC | CTGCGTATGATGGCTCAGATTA |
NC, negative control.
Cell viability assay
Cell viability was evaluated through via Cell Counting Kit-8 (CCK-8) Cell Proliferation and Cytotoxicity Assay Kit (Solarbio, China). Briefly, cells were seeded in 96-well plates at a density of 2,000 cells/well and were incubated for 12, 24, and 48 hours. Subsequently, 10 µL of CCK-8 solution was added per well, and cells were incubated for 1 hour at 37 ℃ in an environment with 5% CO2. Absorbance was measured at 450 nm using the SpectraMax Plus 384 (Molecular Devices, USA).
EdU assay
The BeyoClic EdU Cell Proliferation Kit with Alexa Fluor 555 (Beyotime, China) was used. Cells were initially seeded onto poly-D-lysine (PDL)-coated cell slides at a density of approximately 60%. After treatment, the EdU working solution was introduced, and cells were subsequently incubated for 2 hours at 37 ℃ in an environment with 5% CO2. Cells were fixed with 4% paraformaldehyde for a duration of 30 minutes and permeabilized for 15 minutes. Subsequently, the Click Additive Solution was administered, and the cells were incubated for an additional 30 minutes in darkness. Nuclei were labeled with 4',6-diamidino-2-phenylindole (DAPI). Observations were made using an ECLIPSE Ti-2 fluorescence microscope (Nikon, Japan) with Alexa Fluor 555 (Ex-555 nm and Em-565 nm) and Hoechst 33342 (Ex-346 nm and Em-460 nm).
Colony formation assay
For the colony formation assay, a total of 1,000 cells were initially plated in six-well cell culture plates and maintained at 37 ℃ in a 5% CO2 environment for 10 days. The culture medium was refreshed every 3 days, and the cells were subsequently fixed with 4% paraformaldehyde (Beyotime) for 30 minutes, stained with crystal violet staining solution (Beyotime) for an additional 30 minutes, and rinsed with tap water. The quantification of cell colonies was conducted through manual enumeration, with each experimental condition being repeated three times.
Cell migration and invasion assays
Tumor cell migration and invasion were determined using 8 µm-pore Transwell inserts (Corning, USA). For invasion experiments, 15 µL of Matrigel (BD Biosciences, New Jersey, USA) was added to cells to simulate the internal environment of the tissue (16). Tumor cells were suspended in a serum-free medium and seeded in Transwell chambers at a density of 5×104–10×104 cells/0.2 mL. Transwell inserts were placed in the lower chamber containing 500 µL of complete medium, and cells were then incubated at 37 ℃ in a 5% CO2 environment for a period ranging from 6 to 24 hours. Following this incubation, migrating or invading cells were fixed with 4% paraformaldehyde and subsequently stained with a 0.1% crystal violet solution. Observations were made using an ECLIPSE Ti-2 fluorescence microscope (Nikon, Japan).
Flow cytometry
Cell cycle analysis was performed using a cell cycle and apoptosis detection kit (Beyotime). Briefly, cells were digested with trypsin and harvested, incubated with 1 mL of prechilled 70% ethanol, and fixed at −20 ℃ overnight. After centrifugation, the cells were discarded from the supernatant, mixed with 0.5 mL of the propidium iodide (PI) staining solution (containing RNase A) was added, and then incubated for 30 minutes in the dark and at 37 ℃. Stained cells were analyzed using a CytoFLEX flow cytometer (Beckman Coulter, California, USA).
Cell line-derived xenograft
BALB/c female nude mice, aged 3–4 weeks, were purchased from GemPharmatech Co., Ltd. (Guangzhou, China). An equal number of SK-MES-1 cells were subcutaneously inoculated into nude mice. After 4 weeks, mice were killed and tumor tissues excised. Animal experiments were approved by the Ethics Committee of Sun Yat-sen University (approval No. L102012023060D), in compliance with Regulations for the administration of laboratory animals (2017) guidelines for the care and use of animals. A protocol was prepared before the study without registration.
Fluorescence in situ hybridization (FISH) assay
For FISH analysis of LNCAROD, the LINC01468 probe was synthesized by Ruibiotech (Guangzhou, China). Experiments were performed following the manufacturer’s instructions, and cells were imaged using an FV1000 laser confocal microscope (Olympus, Japan).
Biotin RNA-protein pull-down assay and mass spectrometry analysis
The full-length LNCAROD RNA sequence was transcribed in vitro using the mMESSAGE mMACHINE Kit (Invitrogen, America). Specifically, 10× Biotin RNA labeling Mix (Roche) was used for in vitro biotin labeling of transcripts. Biotinylated transcripts were combined with total lysates from SK-MES-1 cells according to the manufacturer’s instructions. Protein complexes bound to biotin RNA were enriched using streptavidin magnetic beads (Thermo Fisher Scientific). Mass spectrometry analysis was performed by Fitgene Co. Ltd. (Guangzhou, China).
Western blot analysis
Total intracellular protein was extracted using the RIPA buffer (Beyotime) containing protease inhibitors. Protein samples were quantified using a BCA protein concentration assay kit (Beyotime), subjected to separation via sodium dodecyl sulfate-polyacrylamide gel electrophoresis (SDS-PAGE) on 8–12% gels, and transferred onto polyvinylidene difluoride (PVDF) membranes (Merck, Darmstadt, Germany). Samples were treated with 5% skim milk and exposed to primary antibodies overnight at 4 ℃. Subsequently, after exposure to secondary antibodies, luminescence emitted by protein bands was identified with an ECL detection system (Thermo Fisher Scientific). The antibodies used for Western blot analysis are provided in Table 3.
Table 3
Name of antibody | Manufacturers | Article number |
---|---|---|
Anti-Bcl-2 antibody | Abcam | ab194583 |
Anti-cIAP1 antibody | Abcam | ab189193 |
Anti-Bax antibody | Abcam | ab32503 |
AIF Polyclonal antibody | Proteintech | 17984-1-AP |
Anti-ENDOGL1/ENGL antibody | Abcam | ab206300 |
Cytochrome c polyclonal antibody | Proteintech | 10993-1-AP |
Anti-p53 antibody | Abcam | ab32389 |
Anti-p53 (acetyl K120) antibody | Abcam | ab78316 |
Anti-p53 (acetyl K382) antibody | Abcam | ab75754 |
SIRT1 polyclonal antibody | Proteintech | 13161-1-AP |
Anti-DBC-1 (CCAR2) antibody | Abcam | ab215852 |
Anti-RPS19BP1/AROS antibody | Abcam | ab236598 |
Anti-Histone H3 antibody | Abcam | ab201456 |
Anti-GAPDH antibody | Abcam | ab181602 |
Goat anti-rabbit IgG HL (HRP) | Abcam | ab205718 |
WB, western blot.
RNA-binding protein immunoprecipitation
Total RNA was isolated from the cells the TRIzol reagent, which was followed by conjugation of target antibodies or anti-IgG antibodies with protein A/G magnetic beads and incubation with 100 µg of total RNA in an immunoprecipitation buffer containing RNase/protease inhibitors. The eluate was subjected to 2 rounds of elution for 1 hour each at 4 ℃. Subsequently, the resulting products were examined using RT-qPCR to ascertain the presence of protein-enriched RNA. The antibodies employed for the RNA-binding protein immunoprecipitation (RIP) analysis are detailed in Table 4.
Table 4
Name of antibody | Manufacturers | Article number |
---|---|---|
Anti-DBC-1 (CCAR2) antibody | Abcam | ab215852 |
Anti-IGF2BP2/IMP-2 antibody | Abcam | ab128175 |
Rabbit anti-goat IgG HL | Abcam | ab6697 |
Anti-N6-methyladenosine (m6A) antibody | Abcam | ab151230 |
RIP, RNA Binding Protein Immunoprecipitation Assay.
Experimental drugs
Actinomycin D (ACTD) and cycloheximide (CHX) were purchased from Merck. MG132, Inauhzin, and SRT 1720 were purchased from Topscience Co. Ltd. (Shanghai, China).
Agarose gel electrophoresis
TAE buffer was used for the electrophoresis experiments. DNA was separated using 2% agarose gels, electrophoresed in TAE buffer, stained with the SerRed nucleic acid dye (Servicebio, China), and imaged on a Doc XR/ChemiDoc XRS+ Gel Imaging Analysis System (Bio-Rad Laboratories).
Analysis of subcellular segregation
Proteins of the cytoplasmic and nuclear fractions were isolated from cells using NEPER Nuclear and Cytoplasmic Extraction Reagents (Thermo Fisher Scientific). After the cells were lysed in the separation solution, nuclear and cytoplasmic fractions were separated by centrifugation. The expression of target proteins was detected by Western blot analysis, with GAPDH and H3 being used as cytoplasmic and nuclear controls, respectively.
Coimmunoprecipitation (Co-IP) analysis
Cells were harvested and lysed overnight via the addition of the RIPA lysis buffer containing phenylmethanesulfonyl fluoride (PMSF), phosphatase inhibitors, and protease inhibitors, along with 10% SDS and Triton X-100. A small amount of lysis buffer was used for Western blot analysis. After binding to the corresponding antibody, magnetic beads were subjected to binding with total proteins after cell lysis and incubated overnight at 4 ℃, with rotation being applied for coupling. After overnight incubation, magnetic beads were collected using a magnetic bar. The supernatant was carefully removed, and cells were washed 3–4 times with 1 mL of lysis buffer. Finally, 15 µL of 2× SDS loading buffer was added, and the specimens were boiled for 5 minutes. Western blotting was performed to analyze the abundance of the target proteins. Co-IP was performed using antibodies, as shown in Table 5.
Table 5
Name of antibody | Manufacturers | Article number |
---|---|---|
Anti-DBC-1 (CCAR2) antibody | Abcam | ab215852 |
Anti-DDDDK tag (binding to the FLAG tag sequence) antibody | Abcam | ab205606 |
Co-IP, coimmunoprecipitation.
RNA pulse-chase assay
The SK-MES-1 and NCI-H2170 cell lines were subjected to treatment with 5 µg/mL of Actinomycin D for specified durations. Subsequently, total cellular RNA was isolated using TRIzol reagent, which was followed by detection of RNA expression through RT-PCR.
CHX chase assay
SK-MES-1 cells were subjected to treatment with 50 µM of CHX, which was followed by the extraction of cellular proteins via RIPA lysis buffer containing protease inhibitors at specified time intervals. Protein expression was assessed through Western blot analysis.
JC-1 assay
Experiments were performed using the mitochondrial membrane potential assay kit with the JC-1 reagent (Beyotime, China). Cells were seeded in five-well plates, and experiments were performed following the specified protocol. Following removal of the supernatant, 1 mL of cell medium and 1 mL of the JC-1 staining working solution were added, and samples were incubated at 37 ℃ for 20 minutes in a 5% CO2 environment. Samples were incubated with the JC-1 staining buffer and washed twice. Next, 2 mL of the cell culture medium was added and photographed using an ECLIPSE Ti-2 fluorescence microscope (Nikon) with JC-1 monomer (Ex-490 nm and Em-530 nm) and JC-1 polymer (Ex-525 nm and EM-590 nm).
Immunofluorescence assay
Cell slides were washed thrice with Dulbecco’s Phosphate-Buffered Saline (DPBS) for 3 minutes each time. Subsequently, cells were fixed with 4% paraformaldehyde for 2 hours at 4 ℃. Sections were washed three times with PBS for 3 minutes each and incubated with 0.5% Triton X-100 at room temperature (25 ℃) for 20 minutes. The cells were washed three times with PBS for 3 minutes each time. Samples were incubated with goat serum at room temperature for 30 minutes for blocking. Primary antibodies were added to the sections and incubated overnight at 4 ℃. Samples were washed three times with Phosphate-Buffered Saline with Tween-20 (PBST) for 3 minutes each time. Fluorescent secondary antibodies were introduced to the cells, which were then incubated for 1 hour at room temperature. Subsequently, the cells were rinsed three times with PBST for 3 minutes each time, which was followed by quenching with a sealing solution containing DAPI anti-fluorescence quencher. The cells were then observed and photographed under a fluorescence microscope. Antibodies used for immunofluorescence (IF) staining analysis are listed in Table 6.
Table 6
Name of antibody | Manufacturers | Article number |
---|---|---|
Anti-p53 antibody | Abcam | ab32389 |
Goat anti-rabbit IgG HL (Alexa Fluor 488) | Abcam | ab150077 |
IF, immunofluorescence; IgG, immunoglobulin G.
Statistical analysis
Experimental data are presented as the mean ± standard deviation (SD) of at least three independent experiments. The t-test or one-way analysis of variance (ANOVA) was used for comparison between and among multiple groups, respectively. Qualitative data were analyzed using the Chi-squared test. Kaplan-Meier analysis was used for survival analysis, and the log-rank test was used for comparisons between groups. Linear regression analysis was performed to assess the correlation between levels of gene expression. GraphPad Prism 8.0 (GraphPad Software, USA) and SPSS 27 (IBM Corp., USA) were used for statistical analysis. A P value less than 0.05 was considered statistically significant.
Results
LNCAROD was specifically expressed in LUSC and was associated with poor prognosis
Expression data were downloaded from the UCSC Xena database (https://xenabrowser.net/datapages/). Data were screened, and count data of lncRNAs were selected as the input for Differential Expression analysis of RNA-Seq data (DESeq2) for differential gene analysis. The results of the differential analysis were input into Enhanced Volcano for displaying the volcano diagram (Figure 1A). The cellular localization of LNCAROD was identified. DESeq2 was used to analyze the differentially expressed lncRNAs between normal and tumor samples, of which 1,154 were upregulated and 325 were downregulated (q<0.05 and |log fold change| >2). The heat map was plotted with ComplexHeatmap and showed the relative expression of the top 20 upregulated and downregulated genes (Figure 1B). Analysis of The Cancer Genome Atlas (TCGA) database showed that the expression of LNCAROD was significantly upregulated in LUSC tissues relative to the adjacent normal lung tissue (Figure 1C). In addition, 45 pairs of matched LUSCs and normal tissues also indicated significant upregulation of LNCAROD in tumors (Figure 1D). This conclusion was confirmed by RT-qPCR analysis of the clinical samples of LUSC and adjacent normal lung tissues (Figure 1E). Plotting the survival curve using a survival plot indicated that patients with LUSC with low LNCAROD expression had a worse overall prognosis (Figure 1F). To identify the potential function of lncRNA in LUSC, we performed GSEA and found that high expression of LNCAROD was closely related to cancer-related pathways such as EMT, cell junction, P53 pathway, angiogenesis, apoptosis, and hypoxia (Figure 1G). We selected LNCAROD, which is highly expressed in LUSC tissues and demonstrations clinical manifestations of tumor suppression, as our target. Specifically, through examination of the expression of two transcripts (NR_120641.1 and NR_120642.1) in human normal lung epithelial cells (BEAS-2B) and LUSC cells (SK-MES-1, NCI-H2170, NCI-H1703, and NCI-H520), only the transcript NR_120641.1 was found to be highly expressed in LUSC cells (Figure 1H). Compared with the human normal lung epithelial cell line, BEAS-2B, LNCAROD’s expression was upregulated in LUSC cell lines (SK-MES-1, NCI-H2170, NCI-H1703, and NCI-H520) (Figure 1I). The FISH probe for LNCAROD was used to determine its site of expression, and the results showed that LNCAROD expression was mainly localized to the nucleus (Figure 1J). In summary, LNCAROD was specifically expressed in LUSC and negatively correlated with the prognosis of patients with LUSC. Therefore, we inferred that LNCAROD inhibits tumorigenesis and development in LUSC.
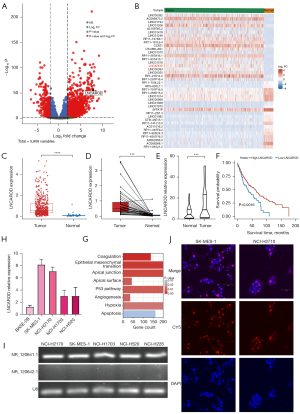
LNCAROD inhibited the growth, migration, and invasion of LUSC cells
A cell line overexpressing LNCAROD (LNCAROD-OE) and that with shRNA interference (LNCAROD-shRNA) were obtained by lentivirus transfection, and the expression of LNCAROD was detected by RT-qPCR (Figure 2A). Stable LNCAROD overexpression and shRNA interference were successfully established in SK-MES-1 and NCI-H2170 cells. Among them, LNCAROD-shRNA1 showed the highest interference efficiency and was used for subsequent experimental studies. The CCK8 assay showed that the activities of SK-MES-1 and NCI-H2170 cells overexpressing LNCAROD were significantly downregulated, while those of SK-MES-1 and NCI-H2170 cells overexpressing LNCAROD were significantly upregulated (Figure 2B). EdU staining showed that the proliferation of SK-MES-1 and NCI-H2170 cells overexpressing LNCAROD was significantly suppressed, while that of SK-MES-1 and NCI-H2170 cells overexpressing LNCAROD was significantly enhanced (Figure 2C). Overexpression of LNCAROD slowed down the rate of cell clone formation, while interfering with LNCAROD expression promoted the formation of cell clones (Figure 2D). Overexpression of LNCAROD could inhibit the migration and invasion of LUSC cells, and interference with LNCAROD expression enhanced the migration and invasion of LUSC cells (Figure 2E,2F). Flow cytometry was performed for cell cycle analysis (Figure 2G). Overexpression of LNCAROD resulted in a slowing down of the cell cycle, decreased the cell proliferation rate, decreased the proportion of cells entering the S phase, and slowed cell division. Interfering with LNCAROD expression increased the cell proliferation rate, the proportion of cells entering the S phase, and the proportion of cells entering the G2 phase. In conclusion, LNCAROD could promote the proliferation and migration of LUSC cells, and its inhibition could promote cell proliferation, growth, migration, and metastasis. Thus, LNCAROD exerts a tumor suppressor effect in LUSC cells.
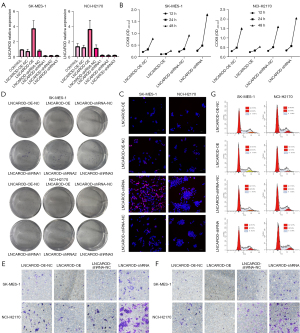
LNCAROD inhibited tumor formation in vivo
To verify the above-mentioned results, SK-MES-1 cells with LNCAROD were injected subcutaneously into nude mice (Figure 3A). After 28 days, tumors were excised, and those in the LNCAROD-OE group were significantly smaller than those in the control group, and tumors in the LNCAORD-shRNA group were significantly larger than those in the control group (Figure 3B). Growth curve analysis and final mass of the tumors in mice confirmed the above results (Figure 3C,3D). Xenograft tumor growth assay in nude mice showed inhibition of tumor growth in the LNCAROD-OE group, while LNCAORD-shRNA cells promoted tumor growth. The extraction of RNA from the tumor tissues for qPCR analysis revealed the expected expression of LNCAROD in the tumor, compared with the NC group, high expression occurred in the OE group and low expression occurred in the shRNA group (Figure 3E). These results indicated that a high expression of LNCAORD could inhibit the occurrence and development of LUSC tumors, and LNCAROD exerted a tumor-suppressing effect in LUSC.
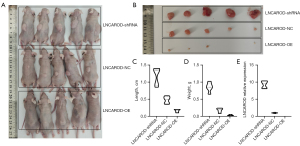
IGF2BP2 regulated the stability of LNCAROD through m6A modification
Through our RNA pull-down and mass spectrometry experiments, IGF2BP2 was found to bind to LNCAROD. Since IGF2BP2 expression was associated with m6A methylation modification, we assessed whether IGF2BP2 mediated the m6A modification of LNCAROD. First, we examined the degree of IGF2BP2 enrichment in the pull-down assay of LNCAROD using Western blotting. IGF2BP2 was found to be bound to LNCAROD (Figure 4A). We performed RIP in SK-MES-1 and NCI-H2170 cells using anti-IGF2BP2, and the products were confirmed by qPCR analysis. LNCAROD was significantly enriched in the RIP product of anti-IGF2BP2 as compared to the IgG group, confirming the binding between IGF2BP2 and LNCAROD (Figure 4B). To verify whether LNCAROD was affected by m6A modification, we performed methylated (Me)RIP-qPCR assays. Knockdown of IGF2BP2 significantly reduced m6A modification of LNCAROD in SK-MES-1 and NCI-H2170 cells (Figure 4C). According to the sequence-based RNA adenosine methylation site predictor (SRAMP) software (http://www.cuilab.cn/sramp), six m6A sites were predicted in LNCAROD (Figure 4D). The mRNA stability assay showed that the knockdown of IGF2BP2 significantly downregulated LNCAROD expression and shortened the half-life of its RNA (Figure 4E). Overall, IGF2BP2 was associated with the m6A modification of LNCAROD and found to promote the stable expression of LNCAROD.
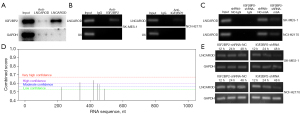
LNCAROD mediated the interaction between CCAR2 and AROS proteins
Based on these results, we explored the molecular mechanism of LNCAROD as a tumor suppressor in the progression of LUSC. Proteins binding to LNCAROD in SK-MES-1 cells were identified by RNA pull-down and mass spectrometry. The combined results of the two showed that CCAR2 and AROS were bound to LNCAROD, which was verified at the protein level by Western blotting (Figure 5A). The results of the RIP assay showed that LNCAROD was precipitated by anti-CCAR2 in SK-MES-1 cells and NCI-H2170 cells (Figure 5B). The cytoplasmic and nuclear fractions of SK-MES-1 and NCI-H2170 cells were isolated by subcellular fractionation, and Western blotting showed that both CCAR2 and AROS proteins were localized in the nucleus (Figure 5C). Analysis of the deletion mutants showed that LNCAROD was bound to CCAR2 in the 1–250 nt region and AROS in the 251–500 nt region (Figure 5D,5E). We confirmed that exogenous and endogenous CCAR2 proteins immunoprecipitated with AROS proteins in SK-MES-1 cells (Figure 5F). Treatment of cell lysates with RNase A significantly reduced the association between CCAR2 and AROS as compared with treatment with RNase inhibitors, indicating the involvement of RNA in facilitating the association between CCAR2 and AROS (Figure 5G). Moreover, interfering with LNCAROD expression in SK-MES-1 cells suppressed the interaction between CCAR2 and AROS, which was enhanced by the overexpression of LNCAROD (Figure 5H). Silencing and overexpression of CCAR2 or AROS had little effect on LNCAROD expression in SK-MES-1 cells (Figure 5I,5J). However, overexpression of LNCAROD promoted CCAR2 expression, and its knockdown inhibited CCAR2 expression. Yet, LNCAROD expression did not affect AROS expression (Figure 5K). Overexpression or knockdown of LNCAROD also did not affect the mRNA levels of CCAR2 and AROS (Figure 5L,5M). LNCAROD was associated with the half-life of CCAR2. Specifically, overexpression of LNCAROD prolonged the half-life of CCAR2, whereas its knockdown shortened it (Figure 5N). The degradation of CCAR2 protein could partially be rescued by treatment of SK-MES-1 and NCI-H2170 with MG132 to knockout LNCAROD (Figure 5O). The loss of LNCAROD accelerated the degradation of the CCAR2 protein. Silencing AROS in SK-MES-1 and NCI-H2170 cells did not reduce the protein and mRNA levels of CCAR2 (Figure 5P,5Q). Therefore, our data confirmed that LNCAROD could stabilize the protein expression of CCAR2 and interact with AROS, but its function should be investigated further.
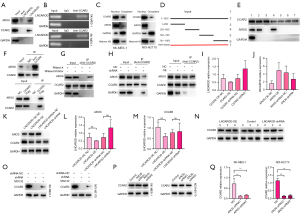
CCAR2 mediated the deacetylation of p53 by inhibiting the activation of SIRT1 by AROS
We experimentally investigated and explored whether the tumor-suppressive function of LNCAROD in LUSC cells is mediated by CCAR2. In SK-MES-1 and NCI-H2170 cells transfected with the LNCAROD-shRNA construct, re-expression of CCAR2 resulted in increased CCAR2 protein levels, increased p53 acetylation, and decreased SIRT1 protein expression. In LUSC cells transfected with LNCAROD-OE, interference of CCAR2 expression resulted in the downregulation of the CCAR2 protein level and p53 acetylation, along with the upregulation of SIRT1 protein expression (Figure 6A). CHX chase assay showed that high LNCAROD expression enhanced p53 stability in LUSC cells, while interference of LNCAROD expression attenuated this stability. Knockdown of CCAR2 expression in LNCAROD-OE cells or overexpression of CCAR2 in LNCAROD-shRNA cells could compensate for this phenomenon (Figure 6B). IF analysis showed that overexpression of both LNCAROD and CCAR2 promoted the expression and cytoplasmic localization of acetylated p53, while interference with LNCAROD and CCAR2 resulted in the opposite (Figure 6C). In LUSC cells transfected with LNCAROD-OE constructs, overexpression of AROS or activation of SIRT1 promoted the downregulation of p53 acetylation and the upregulation of the SIRT1 protein expression. In LUSC cells transfected with LNCAROD-shRNA, interference with AROS expression or inhibition of SIRT1 activity resulted in the promotion of p53 acetylation and downregulation of SIRT1 protein expression (Figure 6D). CHX chase assay showed that overexpression of AROS or activation of SIRT1 promoted p53 degradation and inhibited the effect of LNCAROD-OE on p53 stability. Interference with AROS expression or inhibition of SIRT1 activity could enhance the stability of p53 and inhibit the reduced p53 protein stability due to the knockdown of LNCAROD (Figure 6E). IF staining showed that interference with AROS expression or inhibition of SIRT1 activity could promote the expression and cytoplasmic localization of acetylated p53, while overexpression of AROS or activation of SIRT1 resulted in the opposite (Figure 6F). These data suggest that CCAR2 mediates the effects of AROS and SIRT1 on p53 signaling.
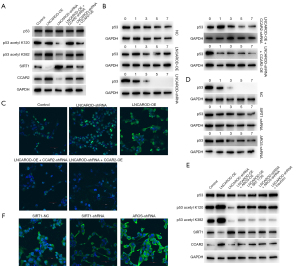
LNCAROD stabilized the expression of CCAR2 and inhibits the proliferation, migration, and invasion of LUSC cells
Functionally, knockdown of CCAR2 in LUSC cells transfected with the LNCAROD-OE construct rescued LUSC cells from reduced cell viability (Figure 7A), colony formation (Figure 7B), and cell proliferation (Figure 7C) and cell cycle arrest (Figure 7D). In contrast, overexpression of CCAR2 in LNCAROD-shRNA-transfected LUSC cells rescued the tumor-promoting effect. Similar results were observed for cell migration and invasion (Figure 7E,7F). Knockdown of CCAR2 rescued reduced cell migration and invasion in LNCAROD-OE-transfected LUSC cells. Overexpression of CCAR2 rescued the upregulation of cell migration and invasion in LNCAROD-shRNA-transfected LUSC cells. Western blot analysis revealed that overexpression of LNCAROD (Figure 7G) resulted in the upregulation of BAX, AIF, ENDOG, and cytochrome C in the cells, concomitant with the downregulation of BCL-2 and c-IAP1. This phenomenon was enhanced by the downregulation of CCAR2. Conversely, the knockdown of LNCAROD with shRNA led to an increase in BCL-2 and c-IAP1 expression, along with a decrease in the levels of BAX, AIF, ENDOG, and cytochrome C. This effect was augmented by the upregulation of CCAR2. JC-1 staining of mitochondria (Figure 7H) revealed a decrease in mitochondrial membrane potential in LNCAROD-OE cells, which was restored following the downregulation of CCAR2 expression. In LNCAROD-shRNA-transfected cells, the mitochondrial membrane potential was slightly enhanced, while it did not change significantly when the expression of CCAR2 was upregulated.
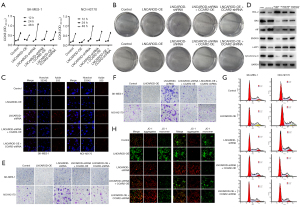
Discussion
In head-and-neck squamous cell carcinoma (HNSCC), m6A methylation is known to enhance the stability of LNCAROD and bind to YBX1 and HSPA1A proteins to preserve the activity of YBX1. Knockdown of LNCAROD reduces cell proliferation and tumorigenicity in vivo, while its overexpression results in enhanced effects (17). In LUAD, SIX5 enhances the expression of LNCAROD. LNCAROD enhances the stability of SERPINE1 mRNA by recruiting SERBP1 and interacts with USP5, thus affecting the ubiquitination of PAI1 protein. The ubiquitination of PAI1 protein in turn promotes the proliferation, migration, and invasion of LUAD cells (18). LNCAROD acts as a conditional factor for enhancing the transcriptional activation of DKK through its nascent spliced released form (19). LNCAROD can enhance the transcription of DKK1 and increase its expression in MCF-7 breast cancer cells through a quasi-cis mechanism (20). As an inhibitor of the WNT signaling pathway, DKK1 is abnormally expressed in tumors, but its function remains controversial. A potential association between methylation of the DKK1 promoter and the development of early-stage tumors in NSCLC, as well as its effect on the prognosis of these patients, has been reported (21). In one study, Kaplan-Meier analysis of cancer stem cells (CSCs) and hepatocellular carcinoma (HCC) revealed that patients with low DKK1 expression exhibited significantly prolonged overall survival and cancer-specific survival compared to patients with high expression. Multivariate analysis further indicated a positive correlation between the mRNA expression of DKK1 and cancer-specific survival rate (22). In our study, we identified LNCAROD as a tumor-suppressor gene involved in the pathogenesis and progression of LUSC. Specifically, in the context of LUSC, LNCAROD exerted its anticancer effects through CCAR2 to inhibit the deacetylation activity of SIRT1. This led to enhanced mitochondrial apoptosis in cells. Consequently, these molecular events resulted in a significant reduction in hallmarks of cancer cells, including proliferation, invasion, metastasis, and tumorigenicity, particularly in LUSC. Importantly, meticulous verification experiments conducted in this study unequivocally demonstrated that there is no discernible association between LNCAROD and previously reported factors such as YBX1, HSPA1A, or PKM.
In the context of tumor cells, the functional role of CCAR2 remains debatable, and its potential as an oncogenic driver or tumor suppressor remains to be definitively elucidated. The interaction between CCAR2 and SIRT1 results in a negative regulation of SIRT1’s function, thereby inhibiting its deacetylase activity (23). SIRT1 (24), an NAD+-dependent deacetylase, can deacetylate both histone and nonhistone proteins. Moreover, SIRT1 deacetylates p53. In U2OS cells, Chk2 facilitates the interaction between CCAR2 and SIRT1, resulting in the suppression of SIRT1 deacetylase activity and the induction of apoptosis through the acetylation of p53. This phenomenon is not contingent upon Thr454 phosphorylation by CCAR2 (25). CCAR2 facilitates the expression of target genes of β-catenin, including PROX1, a transcription factor associated with the progression of CRC. Mechanistically, CCAR2 enhances the interactional stability of LEF1-β-catenin by inhibiting SIRT1-mediated deacetylation of β-catenin, leading to enhanced formation of the LEF1-β-catenin complex and long-range chromatin looping at PROX1 sites. CCAR2 exhibits dual functionality as a co-activator of β-catenin and PROX1 in regulating the β-catenin-PROX1 signaling axis, thereby maintaining the transcriptional activity of PROX1 (26). In the experimental context, LNCAROD expression was positively correlated with the activity of CCAR2, and LNCAROD inhibited the deacetylase activity of SIRT1 by stabilizing CCAR2 and activating CCAR2. The deacetylase activity of SIRT1 is inhibited, resulting in deacetylation inactivation of p53, which undergoes acetylation and is transferred from the nucleus to the outside, triggering the activation of apoptosis.
IGF2BP2 is an integral part of the m6A signaling pathway and plays a crucial role in tumor development. The IGF2BP protein, unlike the proteins of the YTH domain family, serves as an exceptional m6A reader by promoting mRNA stability rather than degradation. Unlike m6A readers containing YTH domains, IGF2BPs exert their effect on target RNAs (including MYC) through an m6A-dependent mechanism, thus enhancing both their stability and expression under normal and stress conditions. This is in contrast to the RNA decay-promoting function of YTH domain-containing family protein 2, which ultimately affects the intricate process of gene expression (27). In CRC cells (28), METTL3 functions as an oncogene and sustains the expression of SOX2 in an m6A-IGF2BP2-dependent manner. In CRC tissues, the expression of SOX2 and both METTL3 and IGF2BP2 are positively correlated. In HCC tissues (29), elevated levels of IGF2BP2 are associated with an unfavorable prognosis, while its overexpression can promote the proliferation of HCC cells in vivo and in vitro. This is attributed to the direct recognition and binding of IGF2BP to the m6A site on FEN1 mRNA, which enhances its stability. Consequently, it is a promising biomarker for predicting the prognoses of patients with HCC. Our results show that LNCAROD stabilizes LNCAROD expression in LUSC by binding to IGF2BP2, and the mechanism is related to the occurrence of m6A in LNCAROD. Stable LNCAROD triggers the molecular mechanism by which CCAR2 in the nucleus inhibits sirt1 activity, leading to the inhibition of sirt1 deacetylation of p53, and finally triggering cell apoptosis based on the transfer of acetylated p53 from the nucleus to the outside of the nucleus.
The p53 protein plays a dual role in cellular growth, serving to maintain the stability of DNA and induction of apoptosis. One study reported that transfection of p53/MDM2-siRNA into SKOV3/DDP cells enhanced their sensitivity to cisplatin and effectively suppressed tumor cell proliferation both in vitro and in vivo (30). At low concentrations of H2O2, p53 stimulates the cellular production of antioxidant enzymes to counteract the toxicity due to reactive oxygen species (ROS). However, at high concentrations of H2O2, the activation of pro-oxidative genes is triggered within cells, indicating that p53 may function as a regulatory switch to induce expressions of distinct genes and elicit diverse stress responses. The transition between these two states is likely affected by the magnitude of the stress stimulus (31). p53 can trigger cell apoptosis in a transcription-independent manner devoid of transactivation of genes (32). Additionally, p53 can directly interact with mitochondria to enhance apoptotic activity (33) or act as a substitute for BH3 proteins. Upon being hindered from entering the nucleus by nuclear transfer inhibitors and forced to express in the cytoplasm, it can induce Bax activation (34). p53 also exhibits diverse cellular functions, with the specific role determined by the type and severity of cellular stress. In our study, despite the presence of an R280K mutation in p53 within SK-MES-1 cells (35), SIRT1-mediated deacetylation of p53R280K or p53 induced mitochondrial apoptosis was not impeded. These findings are promising for the development of future therapeutic interventions targeting p53-mutated LUSC.
Conclusions
The differential expression of LNCAROD was identified as a potential prognostic marker for LUSC. Furthermore, IGF2BP2-mediated m6A methylation was found to stabilize LNCAROD and facilitate CCAR2 in maintaining protein stability. This process inhibits AROS activation of SIRT1, ultimately regulating p53 acetylation and nuclear export to induce mitochondrial apoptosis, which, in turn, could mediate the occurrence and development of LUSC. Based on our findings, we identified LNCAROD as a promising diagnostic marker and endogenous tumor-suppressor gene in LUSC. The elucidation of a regulatory network involving LNCAROD/CCAR2/SIRT1/p53 (Figure 8) provides a more comprehensive means to investigating the pathogenesis and progression of LUSC, along with the associated mechanisms.
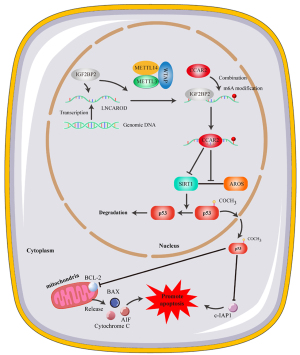
Acknowledgments
We are sincerely grateful to the staff of the Guangdong Esophageal Cancer Institute for their help in completing the project.
Footnote
Reporting Checklist: The authors have completed the ARRIVE and MDAR reporting checklists. Available at https://tlcr.amegroups.com/article/view/10.21037/tlcr-2025-267/rc
Data Sharing Statement: Available at https://tlcr.amegroups.com/article/view/10.21037/tlcr-2025-267/dss
Peer Review File: Available at https://tlcr.amegroups.com/article/view/10.21037/tlcr-2025-267/prf
Funding: This study was supported by
Conflicts of Interest: All authors have completed the ICMJE uniform disclosure form (available at https://tlcr.amegroups.com/article/view/10.21037/tlcr-2025-267/coif). R.B. reports that his institution receives funding from Astra Zeneca, Pfizer, and Roche, and that he serves on the Advisory Board of Bayer, Boeringher Ingelheim, EISAI, Lilly, Menarini, and GSK. The other authors have no conflicts of interest to declare.
Ethical Statement: The authors are accountable for all aspects of the work in ensuring that questions related to the accuracy or integrity of any part of the work are appropriately investigated and resolved. All experiments involving human samples were reviewed and approved by the Ethics Committee of Sun Yat-sen University (approval No. SL-B2023-296-01). This study was conducted in accordance with the Declaration of Helsinki and its subsequent amendments. For this type of study, formal consent was not required, as many of the participants died when the study was initiated. Animal experiments were approved by the Ethics Committee of Sun Yat-sen University (approval No. L102012023060D), in compliance with Regulations for the administration of laboratory animals (2017) guidelines for the care and use of animals.
Open Access Statement: This is an Open Access article distributed in accordance with the Creative Commons Attribution-NonCommercial-NoDerivs 4.0 International License (CC BY-NC-ND 4.0), which permits the non-commercial replication and distribution of the article with the strict proviso that no changes or edits are made and the original work is properly cited (including links to both the formal publication through the relevant DOI and the license). See: https://creativecommons.org/licenses/by-nc-nd/4.0/.
References
- Riely GJ, Wood DE, Ettinger DS, et al. Non-Small Cell Lung Cancer, Version 4.2024, NCCN Clinical Practice Guidelines in Oncology. J Natl Compr Canc Netw 2024;22:249-74. [Crossref] [PubMed]
- Bray F, Laversanne M, Sung H, et al. Global cancer statistics 2022: GLOBOCAN estimates of incidence and mortality worldwide for 36 cancers in 185 countries. CA Cancer J Clin 2024;74:229-63. [Crossref] [PubMed]
- Zhong H, Wang L, Zhu X, et al. STAT3 inhibitor Stattic Exhibits the Synergistic Effect with FGFRs Inhibitor Erdafitinib in FGFR1-positive Lung Squamous Cell Carcinoma. J Cancer 2024;15:5415-24. [Crossref] [PubMed]
- Zhang S, Liu L, Shi S, et al. Bidirectional Association Between Cardiovascular Disease and Lung Cancer in a Prospective Cohort Study. J Thorac Oncol 2024;19:80-93. [Crossref] [PubMed]
- Herbst RS, Morgensztern D, Boshoff C. The biology and management of non-small cell lung cancer. Nature 2018;553:446-54. [Crossref] [PubMed]
- Huang W, Xiong T, Zhao Y, et al. Computational prediction and experimental validation identify functionally conserved lncRNAs from zebrafish to human. Nat Genet 2024;56:124-35. [Crossref] [PubMed]
- Bridges MC, Daulagala AC, Kourtidis A. LNCcation: lncRNA localization and function. J Cell Biol 2021;220:e202009045. [Crossref] [PubMed]
- Lin S, Zhang R, An X, et al. LncRNA HOXA-AS3 confers cisplatin resistance by interacting with HOXA3 in non-small-cell lung carcinoma cells. Oncogenesis 2019;8:60. [Crossref] [PubMed]
- Zong S, Dai W, Guo X, et al. LncRNA-SNHG1 promotes macrophage M2-like polarization and contributes to breast cancer growth and metastasis. Aging (Albany NY) 2021;13:23169-81. [Crossref] [PubMed]
- Lin J, Liao S, Liu Z, et al. LncRNA FGD5-AS1 accelerates cell proliferation in pancreatic cancer by regulating miR-520a-3p/KIAA1522 axis. Cancer Biol Ther 2021;22:257-66. [Crossref] [PubMed]
- Qiu Y, Man C, Zhu L, et al. R-loops' m6A modification and its roles in cancers. Mol Cancer 2024;23:232. [Crossref] [PubMed]
- Yang H, Hu Y, Weng M, et al. Hypoxia inducible lncRNA-CBSLR modulates ferroptosis through m6A-YTHDF2-dependent modulation of CBS in gastric cancer. J Adv Res 2022;37:91-106. [Crossref] [PubMed]
- Fang D, Ou X, Sun K, et al. m6A modification-mediated lncRNA TP53TG1 inhibits gastric cancer progression by regulating CIP2A stability. Cancer Sci 2022;113:4135-50. [Crossref] [PubMed]
- Zhou L, Jiang J, Huang Z, et al. Hypoxia-induced lncRNA STEAP3-AS1 activates Wnt/β-catenin signaling to promote colorectal cancer progression by preventing m(6)A-mediated degradation of STEAP3 mRNA. Mol Cancer 2022;21:168. [Crossref] [PubMed]
- Subramanian A, Tamayo P, Mootha VK, et al. Gene set enrichment analysis: a knowledge-based approach for interpreting genome-wide expression profiles. Proc Natl Acad Sci U S A 2005;102:15545-50. [Crossref] [PubMed]
- Li J, Wang W, Chen S, et al. FOXA1 reprograms the TGF-β-stimulated transcriptional program from a metastasis promoter to a tumor suppressor in nasopharyngeal carcinoma. Cancer Lett 2019;442:1-14. [Crossref] [PubMed]
- Ban Y, Tan P, Cai J, et al. LNCAROD is stabilized by m6A methylation and promotes cancer progression via forming a ternary complex with HSPA1A and YBX1 in head and neck squamous cell carcinoma. Mol Oncol 2020;14:1282-96. [Crossref] [PubMed]
- Yuan Y, Zhou D, Chen F, et al. SIX5-activated LINC01468 promotes lung adenocarcinoma progression by recruiting SERBP1 to regulate SERPINE1 mRNA stability and recruiting USP5 to facilitate PAI1 protein deubiquitylation. Cell Death Dis 2022;13:312. [Crossref] [PubMed]
- Ntini E, Louloupi A, Liz J, et al. Long ncRNA A-ROD activates its target gene DKK1 at its release from chromatin. Nat Commun 2018;9:1636. [Crossref] [PubMed]
- Zhou XL, Qin XR, Zhang XD, et al. Downregulation of Dickkopf-1 is responsible for high proliferation of breast cancer cells via losing control of Wnt/beta-catenin signaling. Acta Pharmacol Sin 2010;31:202-10. [Crossref] [PubMed]
- Stewart DJ. Wnt signaling pathway in non-small cell lung cancer. J Natl Cancer Inst 2014;106:djt356. [Crossref] [PubMed]
- Suda T, Yamashita T, Sunagozaka H, et al. Dickkopf-1 Promotes Angiogenesis and is a Biomarker for Hepatic Stem Cell-like Hepatocellular Carcinoma. Int J Mol Sci 2022;23:2801. [Crossref] [PubMed]
- Wang Y, Wang M, Chen J, et al. The gut microbiota reprograms intestinal lipid metabolism through long noncoding RNA Snhg9. Science 2023;381:851-7. [Crossref] [PubMed]
- Jiang T, Liu E, Li Z, et al. SIRT1-Rab7 axis attenuates NLRP3 and STING activation through late endosomal-dependent mitophagy during sepsis-induced acute lung injury. Int J Surg 2024;110:2649-68. [Crossref] [PubMed]
- Wagle S, Park SH, Kim KM, et al. DBC1/CCAR2 is involved in the stabilization of androgen receptor and the progression of osteosarcoma. Sci Rep 2015;5:13144. [Crossref] [PubMed]
- Yu EJ, Kim SH, Kim HJ, et al. Positive regulation of β-catenin-PROX1 signaling axis by DBC1 in colon cancer progression. Oncogene 2016;35:3410-8. [Crossref] [PubMed]
- Huang H, Weng H, Sun W, et al. Recognition of RNA N(6)-methyladenosine by IGF2BP proteins enhances mRNA stability and translation. Nat Cell Biol 2018;20:285-95. [Crossref] [PubMed]
- Li T, Hu PS, Zuo Z, et al. METTL3 facilitates tumor progression via an m(6)A-IGF2BP2-dependent mechanism in colorectal carcinoma. Mol Cancer 2019;18:112. [Crossref] [PubMed]
- Pu J, Wang J, Qin Z, et al. IGF2BP2 Promotes Liver Cancer Growth Through an m6A-FEN1-Dependent Mechanism. Front Oncol 2020;10:578816. [Crossref] [PubMed]
- Gu J, Tang Y, Liu Y, et al. Murine double minute 2 siRNA and wild-type p53 gene therapy enhances sensitivity of the SKOV3/DDP ovarian cancer cell line to cisplatin chemotherapy in vitro and in vivo. Cancer Lett 2014;343:200-9. [Crossref] [PubMed]
- Freire TS, Mori MP, Miranda JNFA, et al. Increased H2O2 levels and p53 stabilization lead to mitochondrial dysfunction in XPC-deficient cells. Carcinogenesis 2021;42:1380-9. [Crossref] [PubMed]
- Liu Y, Su Z, Tavana O, et al. Understanding the complexity of p53 in a new era of tumor suppression. Cancer Cell 2024;42:946-67. [Crossref] [PubMed]
- Zeng Y, He Y, Wang L, et al. Dihydroquercetin improves experimental acute liver failure by targeting ferroptosis and mitochondria-mediated apoptosis through the SIRT1/p53 axis. Phytomedicine 2024;128:155533. [Crossref] [PubMed]
- Seo SU, Woo SM, Lee SG, et al. BAP1 phosphorylation-mediated Sp1 stabilization plays a critical role in cathepsin K inhibition-induced C-terminal p53-dependent Bax upregulation. Redox Biol 2022;53:102336. [Crossref] [PubMed]
- Magrini R, Russo D, Fronza G, et al. The kinetics of p53-binding and histone acetylation at target promoters do not strictly correlate with gene expression after UV damage. J Cell Biochem 2007;100:1276-87. [Crossref] [PubMed]
(English Language Editor: J. Gray)